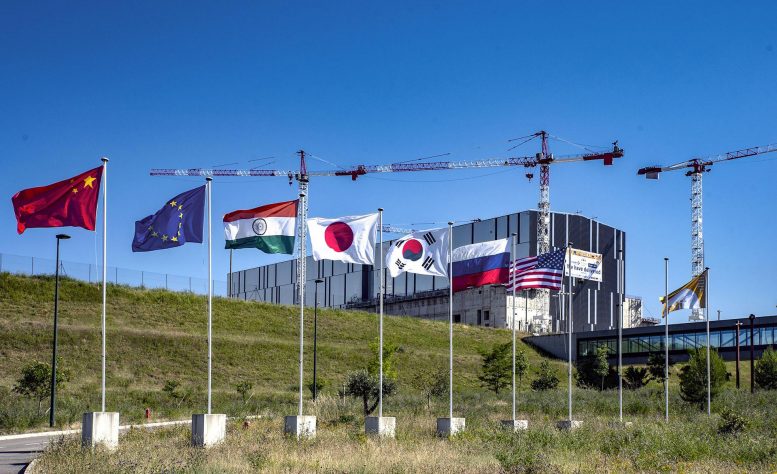
Now 75% completed, ITER under construction in southern France is a collaboration of 35 partner countries: the European Union (plus the United Kingdom and Switzerland), China, India, Japan, Korea, Russia and the United States. Most of ITER’s funding is in the form of contributed components. Credit: ITER
Major milestone in US contribution to ITER.
After a decade of design and fabrication, General Atomics is ready to ship the first module of the Central Solenoid, the world’s most powerful magnet. It will become a central component of ITER, a machine that replicates the fusion power of the Sun. ITER is being built in southern France by 35 partner countries.
ITER’s mission is to prove energy from hydrogen fusion can be created and controlled on earth. Fusion energy is carbon-free, safe, and economic. The materials to power society with hydrogen fusion for millions of years are readily abundant.
Despite the challenges of Covid-19, ITER is almost 75 percent built. For the past 15 months, massive first-of-a-kind components have begun to arrive in France from three continents. When assembled together, they will make up the ITER Tokamak, a “sun on earth” to demonstrate fusion at industrial scale.
ITER is a collaboration of 35 partner countries: the European Union (plus the United Kingdom and Switzerland), China, India, Japan, Korea, Russia and the United States. Most of ITER’s funding is in the form of contributed components. This arrangement drives companies like General Atomics to expand their expertise in the futuristic technologies needed for fusion.
The Central Solenoid, the largest of ITER’s magnets, will be made up of six modules. It is one of the largest of the U.S. contributions to ITER.
Fully assembled, it will be 18 meters (59 feet) tall and 4.25 meters (14 feet) wide, and will weigh a thousand tons. It will induce a powerful current in the ITER plasma, helping to shape and control the fusion reaction during long pulses. It is sometimes called the “beating heart” of the ITER machine.
How powerful is the Central Solenoid? Its magnetic force is strong enough to lift an aircraft carrier 2 meters (6 feet) into the air. At its core, it will reach a magnetic field strength of 13 Tesla, about 280,000 times stronger than the earth’s magnetic field. The support structures for the Central Solenoid will have to withstand forces equal to twice the thrust of a space shuttle lift-off.
Earlier this year, General Atomics (GA) completed final testing of the first Central Solenoid module. This week it will be loaded onto a special heavy transport truck for shipment to Houston, where it will be placed on an ocean-going vessel for shipment to southern France.
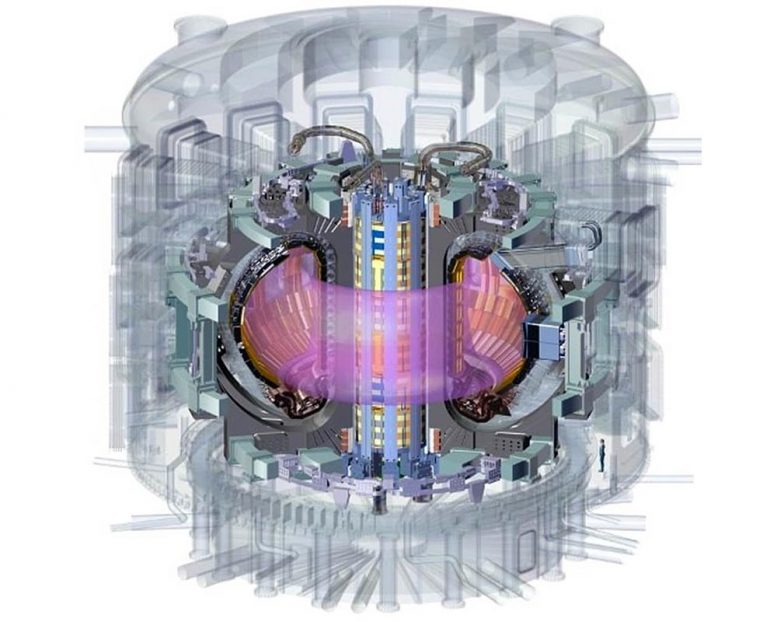
The central solenoid is at the heart of the ITER tokamak. It both initiates plasma current and drives and shapes the plasma during operation. Credit: US ITER
The Central Solenoid will play a critical role in ITER’s mission to establish fusion energy as a practical, safe and inexhaustible source of clean, abundant and carbon-free electricity.
“This project ranks among the largest, most complex and demanding magnet programs ever undertaken,” says John Smith, GA’s Director of Engineering and Projects. “I speak for the entire team when I say this is the most important and significant project of our careers. We have all felt the responsibility of working on a job that has the potential to change the world. This is a significant achievement for the GA team and US ITER.”
The Central Solenoid modules are being manufactured at GA’s Magnet Technologies Center in Poway, California, near San Diego, under the direction of the US ITER project, managed by Oak Ridge National Laboratory (ORNL). Five additional Central Solenoid modules, plus one spare, are at various stages of fabrication. Module 2 will be shipped in August.
The Promise of Fusion
Hydrogen fusion is an ideal method of generating energy. The deuterium fuel is readily available in seawater, and the only by-product is helium. Like a gas, coal, or fission plant, a fusion plant will provide highly concentrated, baseload energy around the clock. Yet fusion produces no greenhouse gas emissions or long-lived radioactive waste. The risk of accidents with a fusion plant is very limited — if containment is lost, the fusion reaction simply stops.
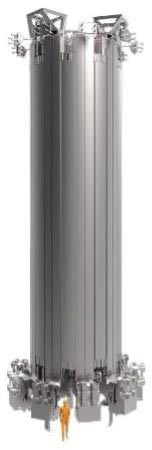
The Central Solenoid, the largest of ITER’s magnets, will be made up of six modules. It is one of the largest of the U.S. contributions to ITER. Credit: ITER / General Atomics
Fusion energy is closer than many people realize. It could provide a source of carbon-free electricity for the grid, playing a key role as the U.S. and other nations decarbonize their generation infrastructure. Two recent reports released by the fusion community lay out ways the U.S. can get there.
In December, the U.S. Department of Energy Fusion Energy Sciences Advisory Committee released a report that lays out a strategic plan for fusion energy and plasma science research over the next decade. It calls for the development and construction of a fusion pilot plant by 2040.
In February of this year, the National Academies of Sciences, Engineering, and Medicine (NASEM) released a complementary report calling for aggressive action to build a pilot power plant. The NASEM report proposes a design by 2028 and a fusion pilot plant in the 2035-2040 timeline.
“The point of working from this timeline was to outline what it would take to have an impact on the transition to reduced carbon emissions by the mid-century. Many investments and essential activities would need to begin now in order to meet that timeline,” says Kathy McCarthy, Director of the US ITER Project Office at Oak Ridge National Laboratory. “The experience we’re gaining from ITER in integrated, reactor-scale engineering is invaluable for realizing a viable, practical path to fusion energy.”
Leveraging global resources for fusion research
ITER (“The Way” in Latin) is one of the most ambitious energy projects ever attempted. In southern France, a coalition of 35 nations is collaborating to build the largest and most powerful tokamak fusion device. The experimental campaign to be carried out at ITER is crucial to preparing the way for the fusion power plants of tomorrow.
Under the 2006 ITER agreement, all members will share equally in the technology developed while funding only a portion of the total cost. The U.S. is contributing about nine percent of ITER’s construction costs.
“The ITER project is the most complex scientific collaboration in history,” says Dr. Bernard Bigot, Director-General of the ITER Organization. “Very challenging First-of-a-kind components are being manufactured on three continents over a nearly 10-year period by leading companies such as General Atomics. Each component represents a top-notch engineering team. Without this global participation, ITER would not have been possible; but as a combined effort, each team leverages its investment by what it learns from the others.”
Both the engineering insights and the scientific data generated by ITER will be critical for the U.S. fusion program. As with the other members, the majority of U.S. contributions are in the form of in-kind manufacturing. This approach allows member countries to support domestic manufacturing, create high-tech jobs, and develop new capabilities in private industry.
“Delivery of the first ITER Central Solenoid module is an exciting milestone for the demonstration of fusion energy and also a terrific achievement of U.S. capacity to build very large, high-field, high-energy superconducting magnets,” says Dr. Michael Mauel of Columbia University. “GA’s success in building, testing, and delivering high-field superconducting magnets for fusion energy is a high-tech breakthrough for the U.S. and gives confidence in realizing fusion power in the future.”
“The United States is a vital Member of the ITER project, which they initiated decades ago,” Bigot explains, “General Atomics, with its world-class expertise in both complex manufacturing and precise control of magnetic fields, is a prime example of the remarkable expertise brought to the table by U.S. scientists and engineers.”
ITER will be the first fusion device to produce net energy across the plasma, meaning the fusion reaction will generate more thermal energy than the energy required to heat the plasma. ITER will also be the first fusion device to maintain fusion for long periods of time. ITER will generate 500 megawatts of thermal fusion power, more than thirty times the current record achieved on the JET tokamak in the U.K.
ITER will have many capabilities that go well beyond current tokamaks. Though ITER will not generate electricity, it will be a critical testbed for the integrated technologies, materials, and physics regimes necessary for the commercial production of fusion-based electricity. The lessons learned at ITER will be used to design the first generation of commercial fusion power plants.
“ITER plays a central role in U.S. burning plasma research activities and is the next critical step in the development of fusion energy,” Dr. Mauel says.
The Central Solenoid in context
The Magnet Technologies Center at General Atomics was developed specifically for manufacturing the Central Solenoid — the largest and most powerful pulsed superconducting electromagnet ever constructed — in partnership with US ITER.
Creating the magnetic fields in a tokamak requires three different arrays of magnets. External coils around the ring of the tokamak produce the toroidal magnetic field, confining the plasma inside the vessel. The poloidal coils, a stacked set of rings that orbit the tokamak parallel to its circumference, control the position and shape of the plasma.
In the center of the tokamak, the Central Solenoid uses a pulse of energy to generate a powerful toroidal current in the plasma that flows around the torus. The movement of ions with this current in turn creates a second poloidal magnetic field that improves the confinement of the plasma, as well as generating heat for fusion. At 15 million amperes, ITER’s plasma current will be far more powerful than anything possible in current tokamaks.
The superconductor material used in ITER’s magnets was produced in nine factories in six countries. The 43 kilometers (26.7 miles) of niobium-tin superconductor for the Central Solenoid was manufactured in Japan.
Together, ITER’s magnets create an invisible cage for the plasma that conforms precisely to the metal walls of the tokamak.
Making the Central Solenoid
Fabrication of the first module began in 2015. It was preceded by almost four years of collaboration with experts at US ITER to design the process and tools for fabricating the modules.
Each 4.25-meter (14-foot)-diameter, 110-tonne (250,000-pound) module requires more than two years of precision fabrication from more than 5 kilometers (3 miles) of steel-jacketed niobium-tin superconducting cable. The cable is precisely wound into flat, layered “pancakes” that must be carefully spliced together.
To create the superconducting material inside the module winding, the module must be carefully heat treated in a large furnace, which functions similarly to that of a convection oven found in many kitchens. The benefit of the convection oven is the ability to shorten the overall process while maintaining uniform “cooking” of the module. Inside the furnace, the module spends approximately ten-and-a-half days at 570°C (1,060°F) and an additional four days at 650°C (1200°F). The entire process takes about five weeks.
Following heat treatment, the cable is insulated to ensure that electrical shorts do not occur between turns and layers. During turn insulation, the module needs to be un-sprung without overstraining the conductor, which is now strain-sensitive due to heat treatment.
To perform the wrapping, the turns of the module are stretched like a slinky, allowing the taping heads to wrap the fiberglass/Kapton insulation around the conductor. Once the individual turns are wrapped, the external module surfaces are then wrapped with ground insulation. The ground insulation consists of 25 layers of fiberglass and Kapton sheets. The ground insulation must also tightly fit around complex coil features, such as the helium inlets.
After insulation, the module is enclosed in a mold, and 3,800 liters (1,000 gallons) of epoxy resin are injected under vacuum, to saturate the insulation materials and prevent bubbles or voids. When hardened at 650°C (260°F), the epoxy fuses the entire module into a single structural unit.
The finished module is subjected to a series of demanding tests, placing it in the extreme conditions it will experience during ITER operation, including near-complete vacuum and cryogenic temperatures required for the magnet to become superconducting (4.5 Kelvin, which equates to about -450°F or -270°C).
Lessons learned on the first Central Solenoid module have been applied to the fabrication of the subsequent six coils.
“For those of us who have dedicated our careers to fusion research, this is undeniably an exciting moment,” said Dr. Tony Taylor, GA’s vice president for magnetic fusion energy. “When the module leaves for its trip to France, we will all be able to take pride in a very significant contribution on the road to fusion energy.”
Shipment to France
ITER construction involves more than 1 million components, manufactured around the world. Many of these components are very large, and the Central Solenoid modules are among the heaviest. The shipping process for the massive magnets requires specialized heavy transport vehicles. The entire process for safely loading and securing the module on the truck, including preparations for lifting, will take about a week.
After loading, the module will be shipped to Houston, Texas, where it will be placed onto a ship for transport to the ITER site. The first module will head to sea in late July and arrive in France in late August. Ground transit to the ITER site will take place in early September.
“Fusion has the potential to provide safe, environmentally friendly energy as a realistic replacement for fossil fuels during this century,” Bigot says. “With a nearly unlimited global supply of fuel, it also has the potential — in complement with renewable energies — to transform the geopolitics of energy supply. I can think of no better illustration of that transformative action than the ITER project, where our U.S. partners work in close collaboration with contributors from China, Europe, India, Japan, South Korea, and Russia, as a single team dedicated to achieving the common goal of a bright energy future.”
How does fusion work?
A small amount of deuterium and tritium (hydrogen) gas is injected into a large, donut-shaped vacuum chamber, called a tokamak. The hydrogen is heated until it becomes an ionized plasma, which looks like a cloud. Giant superconducting magnets, integrated with the tokamak, confine and shape the ionized plasma, keeping it away from the metal walls. When the hydrogen plasma reaches 150 million degrees Celsius — ten times hotter than the core of the Sun — fusion occurs. In the fusion reaction, a tiny amount of mass is converted to a huge amount of energy (E=mc2). Ultra-high-energy neutrons, produced by fusion, escape the magnetic field and hit the metal tokamak chamber walls, transmitting their energy to the walls as heat. Some neutrons react with lithium in the metal walls, creating more tritium fuel for fusion. Water circulating in the tokamak walls receives the heat and is converted to steam. In a commercial reactor, this steam will drive turbines to produce electricity. Hundreds of tokamaks have been built, but ITER will be the first to achieve a “burning” or largely self-heating plasma.
About General Atomics
Since the dawn of the atomic age, General Atomics innovations have advanced the state of the art across the full spectrum of science and technology — from nuclear energy and defense to medicine and high-performance computing. Behind a talented global team of scientists, engineers, and professionals, GA’s unique experience and capabilities continue to deliver safe, sustainable, economical, and innovative solutions to meet growing global demands. GA’s portfolio of innovative programs includes remotely piloted aircraft, such as the Predator and Reaper; the Electromagnetic Aircraft Launch System (EMALS) being installed on the new Ford-class aircraft carriers; and a variety of advanced manufacturing programs that are driving innovation in the nation’s Inertial Confinement Fusion program.
GA has worked in fusion energy since the company’s founding in the 1950s. GA currently operates the DIII-D National Fusion Facility for the U.S. Department of Energy (DOE) Office of Science, where researchers from GA and more than 100 institutions worldwide work to develop the physics basis for practical fusion-generated power.
About US ITER
Dr. Kathy McCarthy is the Director of the US ITER Project Office and the Associate Laboratory Director for Fusion and Fission Energy and Science at Oak Ridge National Laboratory. US ITER is funded by the DOE Office of Science’s Fusion Energy Sciences program. UT-Battelle manages ORNL for the Department of Energy’s Office of Science, the single largest supporter of basic research in the physical sciences in the United States. The Office of Science is working to address some of the most pressing challenges of our time.
About ITER
ITER — designed to demonstrate the scientific and technological feasibility of fusion power — will be the world’s largest experimental fusion facility. Fusion is the process that powers the Sun and the stars: when light atomic nuclei fuse together to form heavier ones, a large amount of energy is released. Fusion research is aimed at developing a safe, abundant and environmentally responsible energy source.