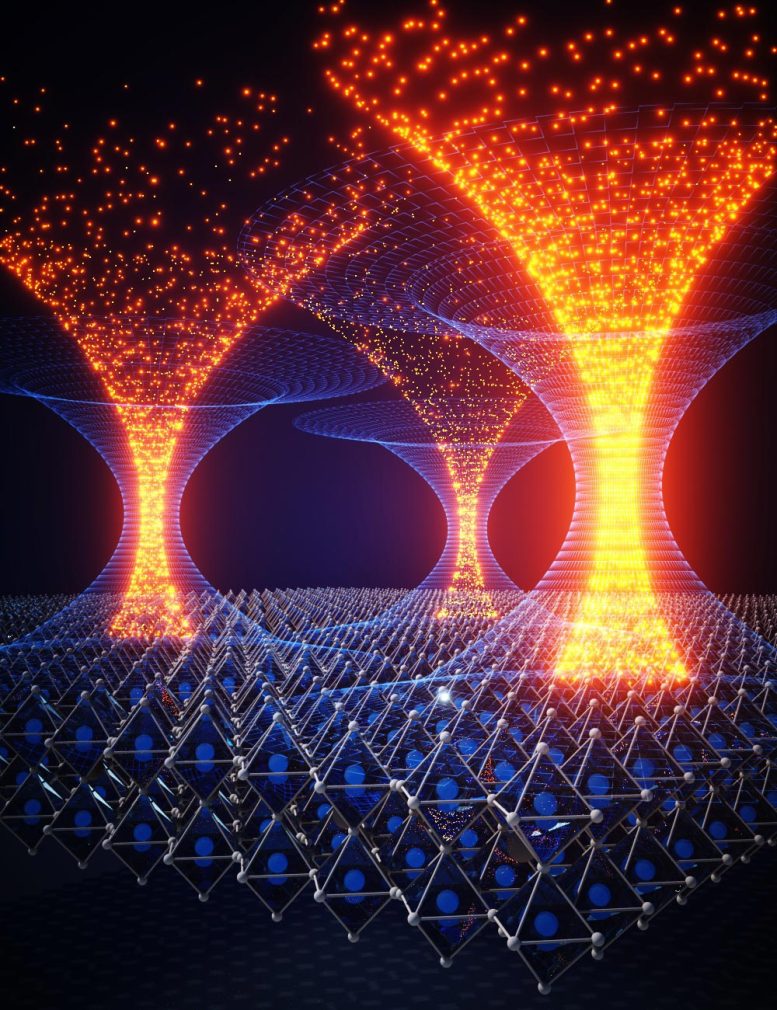
Artistic representation of electrons funneling into high-quality areas of perovskite material. Credit: Alex T. at Ella Maru Studios
Researchers from the University of Cambridge have used a suite of correlative, multimodal microscopy methods to visualize, for the first time, why perovskite materials are seemingly so tolerant of defects in their structure. Their findings were published today (November 22, 2021) in Nature Nanotechnology.
The most commonly used material for producing solar panels is crystalline silicon, but to achieve efficient energy conversion requires an energy-intensive and time-consuming production process to create the highly ordered wafer structure required.
In the last decade, perovskite materials have emerged as promising alternatives.
The lead salts used to make them are much more abundant and cheaper to produce than crystalline silicon, and they can be prepared in a liquid ink that is simply printed to produce a film of the material. They also show great potential for other optoelectronic applications, such as energy-efficient light emitting diodes (LEDs) and X-ray detectors.
The impressive performance of perovskites is surprising. The typical model for an excellent semiconductor is a very ordered structure, but the array of different chemical elements combined in perovskites creates a much ‘messier’ landscape.
This heterogeneity causes defects in the material that lead to nanoscale ‘traps’, which reduce the photovoltaic performance of the devices. But despite the presence of these defects, perovskite materials still show efficiency levels comparable to their silicon alternatives.
In fact, earlier research by the group has shown the disordered structure can actually increase the performance of perovskite optoelectronics, and their latest work seeks to explain why.
Combining a series of new microscopy techniques, the group present a complete picture of the nanoscale chemical, structural and optoelectronic landscape of these materials, that reveals the complex interactions between these competing factors and ultimately, shows which comes out on top.
“What we see is that we have two forms of disorder happening in parallel,” explains PhD student Kyle Frohna, “the electronic disorder associated with the defects that reduce performance, and then the spatial chemical disorder that seems to improve it.
“And what we’ve found is that the chemical disorder – the ‘good’ disorder in this case – mitigates the ‘bad’ disorder from the defects by funneling the charge carriers away from these traps that they might otherwise get caught in.”
In collaboration with Cambridge’s Cavendish Laboratory, the